About in silico medicine
The definition, history, institutions and main achievements of in silico medicine.
Definition
In biology, studies can be conducted in vivo (Latin for ‘within the living’), on whole, living organisms, whether plants, animals or humans, in contrast to in vitro, on living matter – microorganisms, cells, tissues, organs – inside an artificial confinement, whether test tube, culture dish or incubator.
Computer chips are made of silicon, so in silico, ‘within the silicon’, indicates studies conducted using computer modelling and simulation.
In silico medicine (also known as ‘computational medicine’) indicates modelling and simulation technologies that directly contribute to the prevention, diagnosis, prognosis, treatment planning and execution, or management of disease.
In silico medicine technologies provide subject-specific predictions of quantities that are difficult or impossible to measure directly, but which are important to support the medical decisions about a patient.
For example, a subject-specific computer model generated from Magnetic Resonance Imaging data can much more accurately predict if a person is affected by pulmonary hypertension.
Another subject-specific computer model based on fluoroscopy images can provide information essential to deciding the best course of treatment for patients with coronary stenosis, which normally can be measured only with an invasive procedure that few hospitals in the UK can perform.
History
Physiology has a long tradition, dating particularly from the work of Claude Bernard in the 19th century, of quantitative research on the structure–function relationships that underpin physiological processes and the practice of medicine.
This tradition emphasised the integration of multidisciplinary knowledge by using physical laws and mathematics, albeit at a simple level, to understand the complex processes of life. With the elucidation of the genetic and molecular basis of life, however, biomedical research in the latter half of the 20th century largely moved away from physiology toward molecular biology.
Starting in the early 1990s, biomedical researchers started to advocate against the excessive reductionism of molecular biology, noting that it completely neglected the complex interactions between cells, tissues and organs, as well as the roles played by lifestyle, nutrition and the environment.
In 1993, the International Union of Physiological Sciences (IUPS) recognised this dichotomy and established the Physiome Project to introduce engineering approaches and technologies to the physiological sciences. The project developed into a framework for computational physiology that is still being refined.
During its Sixth Framework Programme for Research and Technological Development (FP6, 2002–2006), the European Commission (EC) funded projects in which computational physiology, biophysics, and biomechanics methods were used to address clinically relevant problems.
Yet despite the available expertise, there was a feeling that Europe was ‘missing the boat’: The IUPS had formally endorsed the IUPS Physiome Project in 1993, but the drive was primarily in New Zealand, Japan, and the United States.
Also, in April 2003, the US Interagency Modeling and Analysis Group (IMAG) was formed. It coordinated program staff from the National Institutes of Health (NIH) and the National Science Foundation (NSF) which managed projects in this growing area.
On 1 June 2005, a small group of researchers met officers from the EC in an expert workshop in Barcelona. Following this meeting, in November, a white paper (PDF, 533KB) was published in which, for the first time, the term Virtual Physiological Human (VPH) was used.
In 2007 over 200 experts from all over the world contributed to the report ‘Seeding the Europhysiome’ (PDF, 3.11MB), a research roadmap that set the scene for the development of the VPH.
In 2008 PricewaterhouseCoopers publish a report entitled ‘Pharma 2020: Virtual R&D – Which path will you take?’ (PDF, 402KB) which suggested that the current pharmaceutical industry business model was unsustainable, and that the innovation cycle requires a drastic changes, including the massive adoption of in silico technologies.
In 2010 a constitutional process began that would see established, one year later, the VPH Institute for Integrative Biomedical Research as an international not-for-profit organisation which still today represents this entire research community worldwide.
In 2011 the Policy Affairs Work Group of the VPH Institute produced a position statement on animal experimentation (PDF, 112KB), supporting the thesis that the principles of virtual modelling technologies provided by the VPH could be applied to animal experimentation and so help to reduce the number of animals used in research.
Also, in a position paper on the forthcoming H2020 framework programme, the three targets for in silico medicine technologies are set out:
- The Digital Patient – The VPH for the doctor; patient-specific modelling to support medical decisions. See the Discipulus roadmap (PDF, 10MB) for more details.
- In silico clinical trials – The VPH for the biomedical industry; collections of patient-specific models to augment the preclinical and clinical assessment of new biomedical products; in silico technologies for the reduction, refinement, and partial replacement of animal and human experimentation. See the Avicenna roadmap for more details.
- Personal Health Forecasting – The VPH for the patient/citizen; subject-specific simulations, based on patient data – including those collected by wearable and environmental sensors – that provide advice to individuals affected by conditions requiring self-management or to people at risk of developing disease. See an interview with Professor Viceconti (Former Executive Director of Insigneo) on this topic.
In the autumn of 2011, the development of a new research institute dedicated to VPH research was proposed in Sheffield. In May 2012 the Insigneo institute opened for membership.
The role of in silico medicine within biomedical research
The publication in 1823 of the first issue of the Lancet symbolically marked the moment in the 19th century when the revolution of biomedical research began. Out of this expansion, modern biomedical research has gone on to organise itself around three rather different paradigms, each trying to cope with the impossible complexity of the human body:
- Cellular and molecular biological research, driven by an aggressively reductionist agenda, which focuses on small sub-units of the system.
- Clinical research, which largely treats the human body as a black box, and relies predominantly on the statistical analysis of empirical observations.
- Physiological research, which tries to investigate the human body following the approach typical of the physical and engineering sciences.
The third approach, thwarted by the dramatic limitations of 19th and early 20th century calculus and instrumentation has, until recently, been the least successful of the three, and its importance has been little regarded.
Two events, we believe, are changing this scenario.
The first is the dramatic progress that the physical and engineering sciences have driven, around biomedical instrumentation.
Using x-rays, magnetic fields and ultrasound we can now image the inside of the human body with remarkable accuracy; automated chemical analysers, spectroscopes and sequencers are offering a high-throughput biochemistry that opens entirely new possibilities; the amazing capabilities of modern electrophysiology gives us details on the workings of the heart, the muscles and the brain; motion capture, dynamometry and wearable sensors offer a detailed view of the biomechanics of human movement.
In short, today we can collect a vast library of quantitative data on each individual patient that describes in significant detail their anatomy, physiology, biochemistry, metabolism, and more.
The second is the astonishing sophistication of computation, thanks to advances in mathematics, computational science and engineering, and modern hardware and software to enhance modelling and simulation.
This development is crucial because, for the first time, we can solve the enormous number of complex mathematical equations that can quantitatively describe many physiological and pathological processes. We now have the means to measure or compute pretty much everything required for the complete assessment of each individual patient.
Yet a challenge with complex living organisms is that they are dramatically entangled, such that the functioning of any one of the parts cannot really be assumed to be independent from all the others.
A large part of biological research sidesteps this problem, citing reductionism, and clinical research bypasses it entirely, by ignoring any attempt at seeking detailed mechanistic explanations. But a biomedical research agenda based on the methods of physical and engineering sciences must face this complexity; and this is possible only if we use mathematical and computational methods to formulate our theories and quantitatively compare their predictions to experimental observations as a primary means of their proof or falsification.
Once a theory emerges that is resistant to disproof, the underlying predictive model can be used to solve clinically relevant problems; many of the grand challenges of modern medicine (prevention, personalisation, participation, that with prediction for the vision of a “P4 medicine” first described by Leroy Hood) would be readily addressed by an increased ability to predict the course of a disease and the effect of different treatment options for any given individual.
Therefore, we believe that in silico medicine is the main conduit through which the great physiologists of the past generation will be ultimately proven right, and a biomedical science based on the methods of physical and engineering science will become more and more successful.
We contend that in silico medicine represents a paradigm shift in the sense proposed by philosopher Thomas Kuhn, ‘a fundamental change in the basic concepts and experimental practices of a scientific discipline’.
Important institutions
- The VPH Institute for integrative biomedical research. This is the not-for-profit international organisation that represents the VPH/in silico medicine research community worldwide.
- The Auckland Bioengineering Institute is home to the IUPS Physiome project. This institute, led by Professor Peter Hunter, remains the most important institution in the field worldwide.
- The National Simulation Resource Physiome at the University of Washington Department of Bioengineering. Jim Bassingthwaighte’s team originated the cardiac physiome initiative, and supports a number of essential technologies, including JSIM, the Java-based simulation system for the construction and operation of quantitative numeric models.
- The Interagency Modeling and Analysis Group (IMAG). Since 2003 this initiative, led by Dr Grace Peng, coordinates all USA federal funding agencies that support modelling and simulation research in life science and biomedical research.
- The Center for Advanced Medical Engineering and Informatics. Initiated in 2004 by the Global Centre of Excellence in in silico medicine and funded by the Japanese government under the direction of Professor Kurachi, this is a reference centre in Japan.
- The Institute for Computational Medicine at John Hopkins University. Natalia Trayanova’s lab is only one of the excellent research groups that are part of this recently established institute.
- Neuromuscular Biomechanics Lab at Stanford University. Scott Delp’s team drives the National Institutes of Health Big Data to Knowledge Mobilize Center of Excellence and the NIH National Center for Simulation in Rehabilitation Research, which develops and maintains the OpenSIM software.
- The Department of Biomedical Engineering at the Technical University of Eindhoven. One of the top biomedical engineering departments in Europe, it includes in its professorial staff several leaders in in silico medicine, including Frans van de Vosse, Cees Oomens, Keita Ito and Dan Bader.
Main achievements
2011
The U.S. Food and Drug Administration (FDA) approves T1DMS the first in silico diabetes type I model as a possible substitute for pre-clinical animal testing for new control strategies in Type 1 Diabetes Mellitus, to be used in artificial pancreas technologies.
2014
The U.S. Food and Drug Administration approves the first clinical technology based on subject-specific modelling: HeartFlow’s FFRCT software for measuring coronary blockages non-invasively.
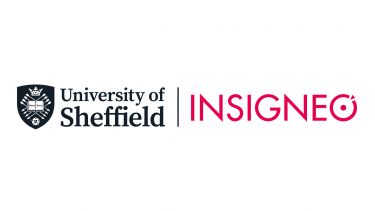